Innovative Approaches to Online Molecular Simulation
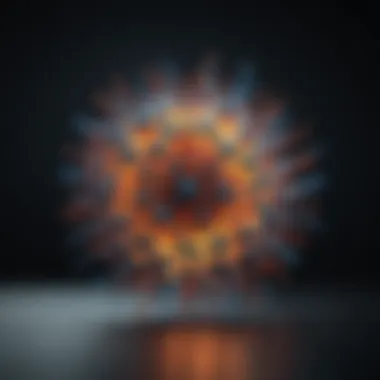
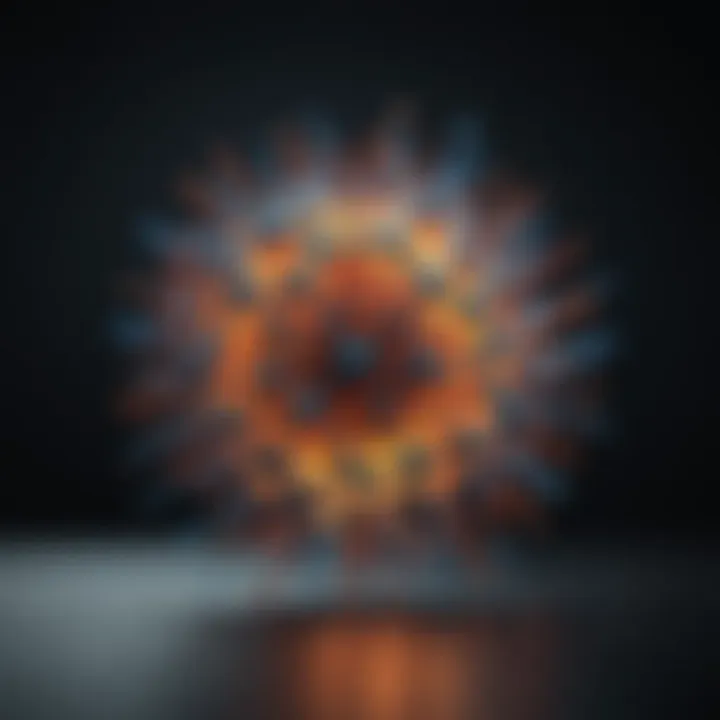
Intro
Molecular simulation has evolved into a central tool for scientific inquiry, spanning various fields such as chemistry, biology, and materials science. This article aims to shed light on the online landscape of molecular simulation. It dives into key methodologies, applications, and future directions, ensuring that both students and professionals gain valuable insights.
The growth of online platforms has democratized access to molecular simulation tools. As a result, researchers can now rapidly analyze complex molecular interactions and apply learned concepts practically. This accessibility has profound implications for education and research, making it essential to understand the techniques and tools available.
Research Overview
Methodological Approaches
To understand the current advancements in molecular simulation, it is vital to explore the core methodologies employed. Two prominent techniques, molecular dynamics and Monte Carlo methods, have laid the foundation for many applications.
- Molecular Dynamics: This method simulates the time-dependent behavior of molecules. It provides insights into structural changes and dynamic processes at an atomic level.
- Monte Carlo Methods: These are statistical approaches that rely on random sampling to explore molecular configurations. This method is beneficial for calculating thermodynamic properties and equilibrium states.
The accessibility of platforms like GROMACS and NAMD online enables users to apply these methodologies effectively. In addition, many educational platforms provide tutorials and simulations that cater to both new learners and experienced researchers.
Significance and Implications
The significance of online molecular simulation lies in its ability to enhance scientific communication and collaboration. Researchers can share findings swiftly through online simulations, facilitating real-time peer reviews and collective problem-solving. The implications are far-reaching, impacting the fields of drug discovery, materials design, and fundamental biophysics research.
"Online molecular simulation opens new avenues for research, transforming how scientists approach problem-solving in various fields."
Furthermore, these tools foster an interdisciplinary approach, allowing professionals from distinct backgrounds to collaborate more effectively. The emphasis on accessibility means that students and educators can engage with cutting-edge research methods, enhancing the learning experience while also preparing future scientists.
Current Trends in Science
Innovative Techniques and Tools
The landscape of molecular simulation is continuously changing, with new techniques and tools emerging regularly. Online platforms now provide user-friendly interfaces for advanced simulations that were once restricted to specialized software.
Some notable tools include:
- OpenMM: A high-performance toolkit that allows simulations of molecular systems.
- Chimera: A visualization tool that aids in analyzing complex molecular structures and dynamics.
Interdisciplinary Connections
The evolving nature of technological advancements in molecular simulation has fostered connections between various scientific disciplines. Fields such as computational biology, medicinal chemistry, and nanotechnology have begun to collaborate closely, sharing resources and methodologies.
The engagement of diverse disciplines enriches research outcomes. This cross-pollination of ideas has led to innovative solutions for complex problems, such as developing new therapeutics or designing novel materials.
Thus the exploration of online molecular simulation not only propels research forward but also encourages an integrated approach to scientific inquiry, enhancing the relevance of findings across multiple fields.
Prelims to Molecular Simulation
Molecular simulation is a crucial aspect of modern scientific research, allowing meticulous exploration of molecular systems. Understanding the underlying processes and their applications is essential for students, researchers, educators, and professionals. These simulations integrate theoretical frameworks and computational techniques to harness insights from molecular behavior, consequently influencing a myriad of fields such as chemistry, biology, and materials science.
Definition and Importance
Molecular simulation encompasses a range of computational techniques used to model and analyze molecular systems at an atomic level. By employing these simulations, researchers can predict molecular properties, understand interactions, and explore potential outcomes in various scenarios without needing to conduct costly or time-consuming experiments. This method is invaluable because it reduces experimental overhead, accelerates research timelines, and facilitates the testing of hypotheses that would otherwise be impractical.
The significance of molecular simulation extends beyond pure research. It drives innovations in drug design, materials engineering, and environmental studies. For example, in pharmacology, molecular simulations help identify potential drug candidates' binding affinities, providing insights into efficacy before clinical trials begin. Consequently, these simulations hold tremendous promise for revolutionizing traditional approaches in scientific inquiry.
Historical Context
The development of molecular simulation can be traced back several decades, with roots in statistical mechanics and quantum dynamics. Early models were simplistic, relying on fundamental principles to predict molecular behavior without extensive computational power. As technology advanced, so too did the complexity of simulations. The introduction of personal computers and specialized software packages enabled a broader audience to access these tools.
Pioneering works in the late 20th century notably propelled molecular simulation forward. The establishment of software like CHARMM and GROMACS laid foundations that many researchers still build upon today. Furthermore, the increasing availability of cloud computing resources has allowed simulations, which once required significant computational time and effort, to be performed on an unprecedented scale.
In summary, the historical evolution of molecular simulation marks a transitional journey from basic theoretical models to sophisticated simulations benefiting numerous scientific realms. Understanding this journey is essential for comprehending current practices and future enhancements in molecular simulations.
Fundamentals of Molecular Simulation
Molecular simulation is a crucial aspect of scientific research that allows professionals to predict and analyze molecular behavior in various situations. Understanding the fundamentals of molecular simulation helps in navigating complex chemical and biological systems. This section focuses on essential principles and techniques, addressing their benefits in research, education, and practical applications.
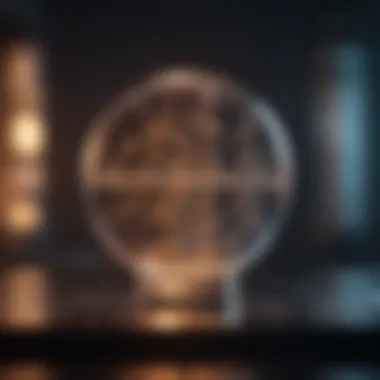
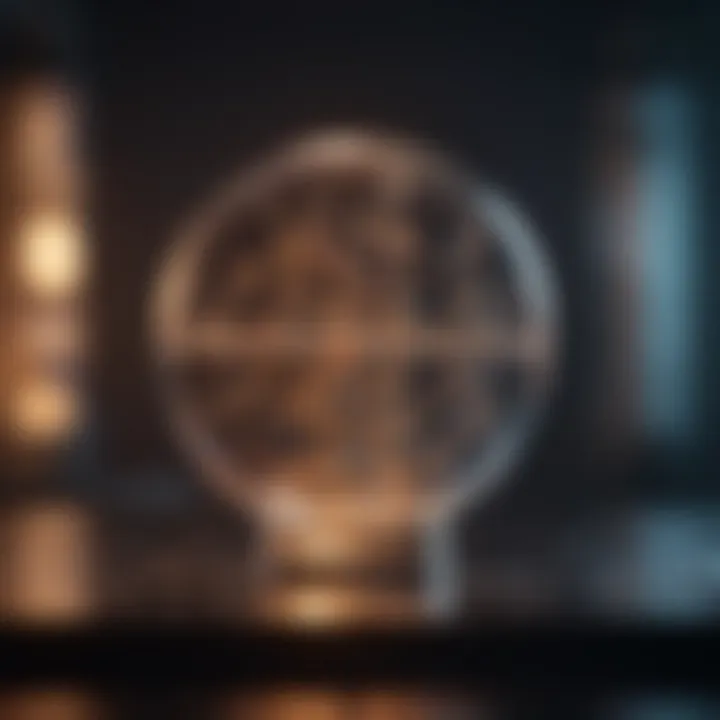
Basic Principles
The basic principles of molecular simulation revolve around modeling the interactions and dynamics of atoms and molecules. These simulations aim to replicate real-world behaviors and situations in a controlled virtual environment. Key principles include:
- Energy Calculations: These simulations often begin with energy calculations to understand the stability and reactivity of molecules involved. Energy minimization helps find the most favorable conformations by reducing high-energy arrangements.
- Physical Laws: Molecular simulations often apply the laws of thermodynamics and statistical mechanics. This ensures that the simulations faithfully reflect the physical reality of molecular interactions.
- Time Evolution: Simulations typically proceed by computing the trajectories of molecules over time. This allows researchers to observe processes such as diffusion, reaction mechanisms, or conformational changes.
Key Techniques
Several key techniques are employed in molecular simulation, each with unique characteristics and applications. Understanding these methods is beneficial for selecting the appropriate approach.
Quantum Mechanics
Quantum mechanics forms the backbone of many molecular simulations, especially in areas like drug design and material science. It offers a detailed view of electron interactions and bond formations. This method is characterized by:
- Precision: Quantum mechanics provides high accuracy in energy calculations, making it a preferred choice for studying small molecular systems.
- Basis Sets: It employs various basis sets that represent wave functions. The choice of basis set can affect accuracy and computational cost.
- Limitations: However, quantum calculations can be computationally expensive and may not scale well for larger systems. This poses practical challenges for researchers.
Classical Mechanics
Classical mechanics simplifies the behavior of molecules by treating them as particles. This technique is beneficial for studying larger systems over extended periods. Its characteristics include:
- Simplicity: Classical mechanics is often easier to compute than quantum mechanics. It uses force fields to calculate interactions between atoms.
- Scalability: It handles large molecular systems efficiently, making it suitable for simulations involving numerous particles compared to quantum methods.
- Limitations: On the downside, it can miss vital quantum mechanical effects. Therefore, it may not be suitable for systems where electronic effects are critical.
Statistical Mechanics
Statistical mechanics bridges the macroscopic and microscopic worlds. It utilizes statistical principles to predict the behavior of molecular systems. Key aspects are:
- Thermodynamics: Statistical mechanics is rooted in thermodynamic principles. It allows for the derivation of macroscopic properties from microscopic behaviors.
- Ensemble Techniques: It employs different ensembles to study systems under various conditions, such as temperature and pressure, enhancing understanding of phase transitions and equilibrium states.
- Computationally Intensive: The computations can become complex, particularly for large systems or over long timescales. Despite this, it provides insights into collective behaviors of molecules that other methods may overlook.
In summary, each fundamental aspect of molecular simulation offers distinct advantages and limitations. Understanding these techniques can significantly influence research outcomes and methodologies.
Molecular Dynamics Simulations
Molecular dynamics simulations play a crucial role in the landscape of molecular simulation, especially when considered in an online context. These simulations provide detailed insights into the behavior of particles at the molecular level, offering a way to visualize and predict molecular interactions over time. By employing classical mechanics, molecular dynamics allows researchers to study the physical movements of atoms and molecules, simulating their dynamics under specified conditions.
One significant benefit of molecular dynamics simulations is their capacity to model complex systems that traditional experimental methods cannot easily explore. This is particularly important in fields such as biochemistry and materials science, where understanding molecular interactions can lead to breakthroughs in drug design and material development. Moreover, the real-time analysis the simulations permit can yield invaluable data, helping researchers observe phenomena that occur at time scales that are otherwise unattainable in laboratory settings.
However, several considerations accompany molecular dynamics simulations. Issues such as computational power requirements and the complexity of the models can pose challenges. Accurately modeling a system requires considerable resources, meaning not all researchers can access the necessary computational capabilities. Therefore, the evolution of online molecular simulations tools aims to democratize access to these valuable resources while still providing high-quality simulation environments.
Overview of Molecular Dynamics
Molecular dynamics involves the numerical simulation of molecular systems, where atoms are thought of as particles interacting through various forces. It provides a powerful framework for investigating various phenomena, from diffusion processes to the dynamics of large biomolecules. Using the principles of classical mechanics, these simulations allow scientists to calculate the trajectories of molecules over time by integrating Newton's equations of motion.
The broad application of molecular dynamics has made it a standard approach in many scientific fields. It has found uses in drug discovery, protein folding studies, and the development of nanomaterials, among others. The adaptability and versatility of molecular dynamics simulations continue to expand, benefiting from advances in algorithms and computational power.
Algorithms Used
Molecular dynamics simulations employ various algorithms to solve the equations governing molecular motion. Two of the prominent algorithms are the Leapfrog Algorithm and the Velocity Verlet Algorithm. Each algorithm has specific characteristics that make it suitable for different simulations.
Leapfrog Algorithm
The Leapfrog Algorithm is notable for its simplicity and efficiency in updating the positions and velocities of particles in simulations. This method uses a staggered time approach, where positions and velocities are computed at alternate time steps. This characteristic gives the algorithm its name because it 'leaps' forward in time.
The benefit of the Leapfrog Algorithm is its numerical stability, especially for long-term simulations. It conserves energy well, which is crucial for simulating isolated systems. However, its unique feature of requiring careful synchronization between position and velocity updates can sometimes lead to challenges in implementation, particularly for beginners in the field.
Velocity Verlet Algorithm
The Velocity Verlet Algorithm is another favored choice among researchers. It is well-known for its direct calculation of both positions and velocities at every time step. This algorithm shares some similarities with the Leapfrog approach but manages to combine both updates in a single computation.
One significant advantage of the Velocity Verlet Algorithm is its strong performance in maintaining stability and accuracy across various time scales. This unique feature makes it particularly useful for simulations where precision is vital, such as studying molecular conformations and thermodynamic properties. However, it may require more computational resources than the Leapfrog Algorithm, which could be a consideration for extensive simulations.
Overall, choosing between these algorithms depends on the specific needs of the simulation and the resources available. Understanding their unique strengths and weaknesses is essential for effective application in molecular dynamics.
Monte Carlo Methods in Molecular Simulation
Monte Carlo methods represent a cornerstone in the realm of molecular simulation. They offer versatile techniques for predicting molecular behavior through stochastic sampling. These methods hinge on randomness, employing a framework that allows the study of systems too complex for analytical solutions. In this section, we will explore the principles behind these methods and examine various applications across disciplines.
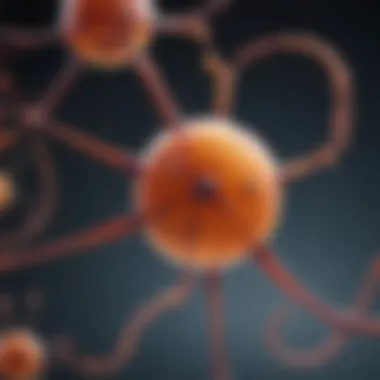
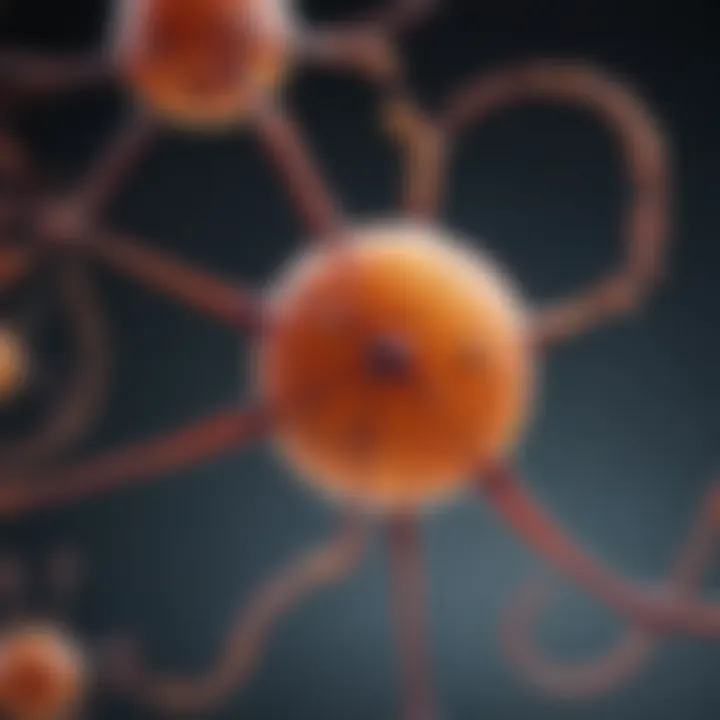
Principles of Monte Carlo Methods
At the heart of Monte Carlo methods lies the principle of random sampling. This approach generates a vast number of configurations for molecular systems, with each configuration representing a potential state of the system. The steps involved in this process include:
- Random Selection: Changes are proposed to the molecular configuration, often termed as trial moves. These changes may involve the rotation or translation of molecules.
- Statistical Weighting: Each configuration is assigned a probability based on the energy of that configuration in comparison to others. The Boltzmann factor is a common method used here, defined as e^-E/kT, where E is the energy, k is the Boltzmann constant, and T is the temperature.
- Acceptance Criterion: Proposed configurations are either accepted or rejected based on their probability. If the configuration improves the system's energy, it is likely accepted. Conversely, a less favorable configuration may still be accepted based on probability, allowing for exploration of energy states beyond the immediate neighborhood.
Through Monte Carlo methods, researchers can estimate thermodynamic properties, such as pressure, energy, and specific heat, with considerable accuracy. The methodologies are particularly well-suited for studying phase transitions and equilibrium properties of complex systems.
Applications and Use Cases
Monte Carlo methods have a broad spectrum of applications in various scientific fields. Here are some notable use cases:
- Biomolecular Simulations: These methods are adept at investigating protein folding and interactions, where complex energy landscapes challenge traditional computational approaches.
- Nanotechnology: Simulations involving nanoparticles can predict interactions with biological systems, offering insights for drug delivery applications.
- Polymer Science: Monte Carlo methods aid in understanding the behaviors of polymers under different conditions, such as temperature changes and external forces.
- Material Science: These methods assist in the design of new materials by predicting properties like strength and thermal conductivity based on molecular configuration.
In addition to these fields, researchers benefit from the ability to utilize online platforms for Monte Carlo simulations, enabling broader access to sophisticated computational techniques without requiring extensive hardware. This aspect of accessibility underscores the relevance of Monte Carlo methods in contemporary research, particularly as the scientific community moves toward more inclusive practices.
"Monte Carlo methods, with their reliance on random sampling, opened a new avenue for exploring molecular dynamics, providing insights that were once considered computationally unattainable."
In summary, the importance of Monte Carlo methods cannot be overstated. They play a significant role in enabling complex molecular simulations that apply across various scientific domains. As online platforms for molecular simulation continue to grow, the impact and utility of these methods are set to expand even further.
Online Platforms for Molecular Simulation
The rise of online platforms for molecular simulation marks a significant development in computational research. These platforms allow students, researchers, and professionals to conduct complex simulations without the need for extensive local resources. They provide immediate access to advanced computational tools and frameworks, enabling users to focus on their research rather than on the underlying hardware or software constraints. This has made molecular simulations more accessible and diverse, which is crucial in a field that requires both rapid advancements and collaboration.
In addition, remote platforms enable researchers from various geographical locations to work together, leveraging each other's expertise. This collaborative environment enhances innovation and facilitates rapid problem-solving by bringing diverse perspectives to the same research question.
Popular Online Tools
Platforms Overview
The platforms available for molecular simulation display a rich variety of capabilities, each suited for different applications. Some popular tools include Galaxy, CHARMM-GUI, and Avogadro. These platforms usually offer user-friendly interfaces and step-by-step tutorials, which help new users navigate complex processes.
One key characteristic of these platforms is their cloud-based architecture. This significantly reduces the computational load on local machines. Rather than relying on personal computers with limited processing power, users can leverage the vast computational resources of the cloud. As a result, educators and students can perform simulations that require substantial computing capabilities, which may not be feasible on personal devices.
Each platform may have unique features. For instance, CHARMM-GUI excels in its ability to build complex biomolecular systems, making it attractive for biologists. Users can create simulations of proteins, nucleic acids, and lipids easily. However, the learning curve can be steep for individuals unfamiliar with molecular simulation principles.
Accessibility Features
Accessibility features of online platforms have transformed how users engage with molecular simulations. An important aspect of accessibility is the ease of use for those with limited technical skills. Online tools often provide graphical user interfaces that allow for simpler navigation and operation.
One of the key characteristics of accessibility features is the comprehensive help documentation these platforms offer. Users can refer to user manuals, FAQs, and community forums to seek assistance. This support network can be invaluable for new users starting their journey in molecular simulations.
Additionally, many platforms incorporate language support, enabling non-native English speakers to use the tools effectively. This inclusivity enhances the understanding of molecular simulation techniques and allows broader participation in scientific research. However, while these features improve overall user experience, some advanced functionalities may remain complex, creating a barrier for users trying to maximize the platform's potential.
User Experiences and Feedback
User experiences with online molecular simulation platforms are generally positive but vary widely based on individual needs. Many users appreciate the accessibility of tools and the ability to conduct simulations remotely. Reviews often commend cloud computation for allowing them to avoid technical issues with local hardware.
However, feedback also highlights frustrations, particularly regarding platform limitations. For example, some tools may not support certain types of simulations or fail to deliver results in a time-efficient manner. Users often express a desire for better integration among various tools to streamline workflows. The overall sentiment is one of gratitude for the advancements made possible by these platforms, combined with a call for enhancements in their functionality and performance.
"The ability to run molecular simulations from any computer has changed how I approach my research," one user stated in a forum discussion. "But I still find myself wishing for improved compatibility across different platforms."
Ultimately, the feedback received by online molecular simulation tools emphasizes the need for continuous improvement in both usability and computational efficiency.
Applications of Online Molecular Simulation
The applications of online molecular simulation are vast and multifaceted. They play a crucial role in advancing research and education across various scientific disciplines. These simulations allow for detailed analysis and exploration of molecular interactions that are often not feasible with experimental methods alone.
Biological Applications
In biology, molecular simulations are instrumental in understanding complex biological systems. They aid in the study of protein folding, ligand binding, and molecular interactions within cell membranes. For instance, simulating the structure and dynamics of proteins can provide insights into their function, aiding drug design and development. Researchers can visualize how drugs interact with target proteins, optimizing efficacy and minimizing side effects. Moreover, online platforms enable biologists to access sophisticated tools without the need for extensive computational resources. This accessibility democratizes research, allowing more people to engage in cutting-edge scientific work.
Chemical Applications
Chemical applications of molecular simulation are equally significant. These tools assist chemists in modeling chemical reactions and exploring reaction pathways. They also help predict the properties of new compounds. For example, during the drug discovery process, simulations can be employed to predict how different chemical candidates behave under various conditions. Furthermore, simulations can identify optimal conditions for reactions, enhancing sustainable practices in the chemical industry. By employing online molecular simulation platforms, chemists can work collaboratively across institutions, sharing data and insights in real-time.
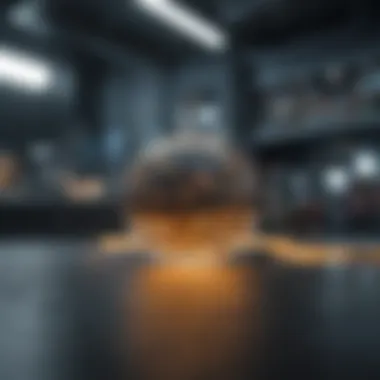
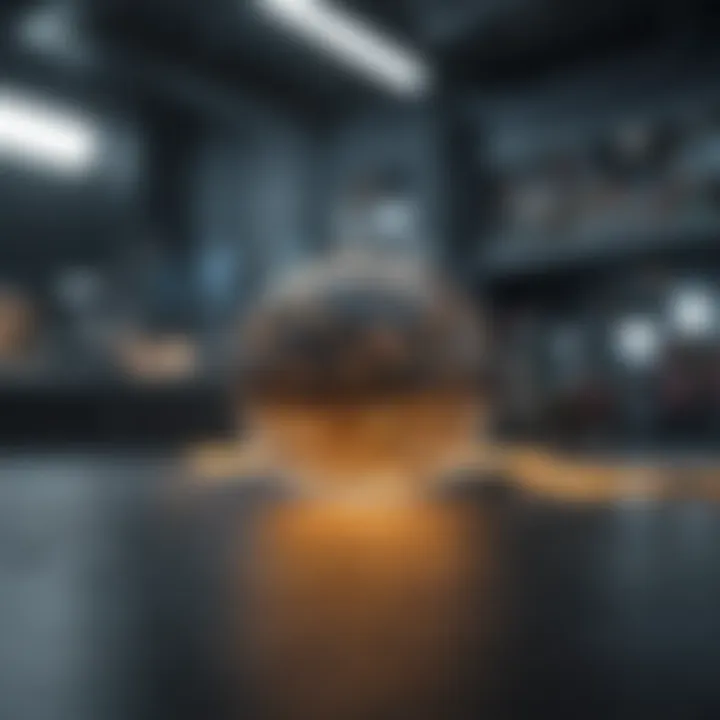
Materials Science Applications
In materials science, molecular simulation contributes to innovation in the design of new materials. Researchers can simulate the behavior of materials at the atomic level, enabling the exploration of different configurations and properties. This approach is particularly beneficial for developing polymers, nanomaterials, and composite materials. Simulations can forecast material stability and performance under different stresses and temperatures. Consequently, researchers can optimize material properties before physical prototyping, considerably saving time and resources.
"Molecular simulation is not just a computational exercise; it is an essential tool for informing experimental design and advancing knowledge in various scientific fields."
In all these fields, online molecular simulation platforms enhance collaboration among researchers, allow for rapid iteration of designs, and facilitate the sharing of findings. As access to these tools becomes more widespread, it is expected that the breadth and depth of applications will only continue to grow.
Challenges in Molecular Simulation
Molecular simulation, despite its vast potential, faces several challenges that impact its effectiveness and application. Understanding these challenges is crucial for researchers, educators, and students in order to navigate the complexities of this field. This section delves into significant obstacles like computational constraints and accuracy and validation issues.
Computational Constraints
One of the major hurdles in molecular simulation is related to computational constraints. These constraints encompass both hardware limitations and software inefficiencies. As simulations involve complex models that estimate the behavior of molecules over time, they require substantial computational power. High-performance computing clusters or supercomputers are often necessary to handle extensive simulations, especially when dealing with large biomolecular complexes or materials at an atomic resolution.
The need for potent hardware results in limitations for many researchers, particularly those in smaller institutions or developing countries. Accessibility to adequate computational resources can hinder research progress. Moreover, excessive computational demand can lead to increased time for obtaining results, thus slowing down the iterative process of experimentation and validation. Strategies to optimize simulations, such as adaptive methods or reducing dimensions of the system, can be explored to ameliorate these challenges but often involve a trade-off between accuracy and computational feasibility.
For example, implementing more efficient algorithms can significantly reduce simulation times, yet might lead to loss in precision. Researchers must carefully consider these trade-offs when designing their experiments.
Accuracy and Validation Issues
Alongside computational constraints, accuracy and validation present ongoing challenges in molecular simulation. The reliability of simulation results is paramount, as they often inform experimental designs and hypothesis testing. Inaccuracies may arise from several sources, such as limitations in the force fields that model interactions or inadequate sampling of configuration space.
Validation is essential to establish the credibility of simulation findings. This process typically involves comparing simulation results with experimental data or established theoretical values. However, discrepancies can occur, making it necessary for researchers to continuously refine their simulation methodologies.
The accuracy of molecular simulations hinges on both the choice of algorithms and the inherent parameters used in the models.
Researchers must be transparent about the limitations of their models and recognize potential biases in their simulations. By leveraging comparison with empirical data and engaging in collaborative validation efforts, the scientific community can collectively progress toward more reliable molecular simulations.
In summary, the challenges in molecular simulation, including computational constraints and accuracy validation, are significant. Addressing these challenges will require innovative solutions and a collaborative effort within the scientific community. Understanding and overcoming these issues will pave the way for more robust applications of molecular simulations across diverse fields.
Future Directions in Molecular Simulation
As the field of molecular simulation evolves, understanding the future directions becomes crucial. This section illuminates multiple paths that molecular simulation is likely to take. It emphasizes innovations that could revolutionize research methodologies and enhance the quality of simulations. By focusing on upcoming technologies and the need for collaborative efforts between disciplines, we can appreciate how these factors will substantially impact future research and applications.
Emerging Technologies
Technological advancements continually reshape molecular simulation. Some critical areas include:
- Machine Learning: This is a growing field that integrates AI to improve predictive modeling. Algorithms can analyze vast data sets for pattern recognition, thus enhancing simulation accuracy.
- Quantum Computing: Quantum mechanics can allow simulations to run more efficiently than classical methods. These enable researchers to simulate larger systems with greater accuracy.
- Cloud Computing: As more simulation tools move online, cloud-based platforms are becoming increasingly relevant. They provide researchers with access to powerful computational resources without needing to invest in expensive hardware.
Each of these technologies brings distinct benefits. Machine learning can significantly reduce computation time, allowing for rapid exploration of chemical space. Quantum computing holds promise for tackling problems that are currently intractable. Finally, cloud-based systems can democratize access to simulation tools for a wider range of users.
Cross-disciplinary Collaborations
Another direction is the growth of cross-disciplinary collaborations. Chemistry, biology, and computer science are traditionally viewed as distinct spheres but can greatly benefit from combined efforts.
Some points to consider include:
- Holistic Approaches: By merging knowledge from various disciplines, researchers can develop more comprehensive models of complex systems. This can lead to breakthroughs in drug design or material science.
- Shared Resources: Cross-disciplinary projects often leverage shared tools and databases. This can streamline research processes and reduce redundancy in efforts.
- Training and Knowledge Transfer: Collaborative initiatives promote skill-sharing. As students work across disciplines, they learn valuable techniques and perspectives they might not encounter in a singular field.
"The convergence of fields will lead to greater innovation and potentially unforeseen solutions to existing problems."
Embracing these future directions not only enriches the field of molecular simulation but also enhances its impact on broader scientific inquiries. As technology progresses and collaboration expands, the implications for research and practical applications only become more significant.
Closure
The conclusion serves as the critical culmination of the insights explored throughout the article about molecular simulation conducted online. This section highlights the importance of understanding molecular simulation not only as a technical practice but also as a significant advancement in research methodology within various scientific disciplines.
In evaluating the trajectory of online molecular simulation, several key elements emerge as particularly vital. Firstly, the accessibility of sophisticated tools and platforms allows a broader range of students, researchers, and educators to engage with and benefit from molecular simulations. This democratization of technology plays a crucial role in fostering innovation.
Secondly, molecular simulation techniques, such as molecular dynamics and Monte Carlo methods, transcend traditional laboratory environments. They empower users to explore complex molecular interactions, allowing for enhanced predictive modeling and analysis without the need for extensive physical resources.
Additionally, the ongoing evolution of these platforms suggests a promising future shaped by emerging technologies and cross-disciplinary collaborations. As new methods and tools continue to be developed, the quality and accuracy of simulations are expected to improve, strengthening their application in real-world scenarios. This bodes well for future breakthroughs in fields ranging from drug design to material science.
However, it is crucial to remain vigilant about the challenges in molecular simulation, including computational constraints and accuracy concerns. Researchers must prioritize validation to ensure that simulations yield reliable and relevant insights. Those efforts contribute to the integrity of findings when applied to practical problems.
"Molecular simulation is not just a tool; it is a conduit for collaboration, innovation, and discovery across disciplines."
In essence, the conclusion emphasizes the intertwined nature of accessibility, technology, and validation in the realm of online molecular simulation. Concerns about the limitations must be balanced with the potential benefits that these simulations present. As the landscape continues to evolve, understanding these dynamics will be essential for harnessing the full potential of molecular simulation in scientific inquiry and discovery. This not only enriches academia but also has the power to drive significant advancements in various industries.