Understanding X-ray Diffraction Principles and Applications
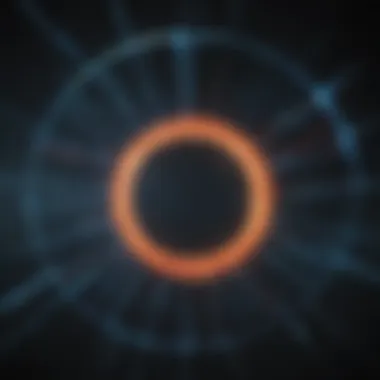
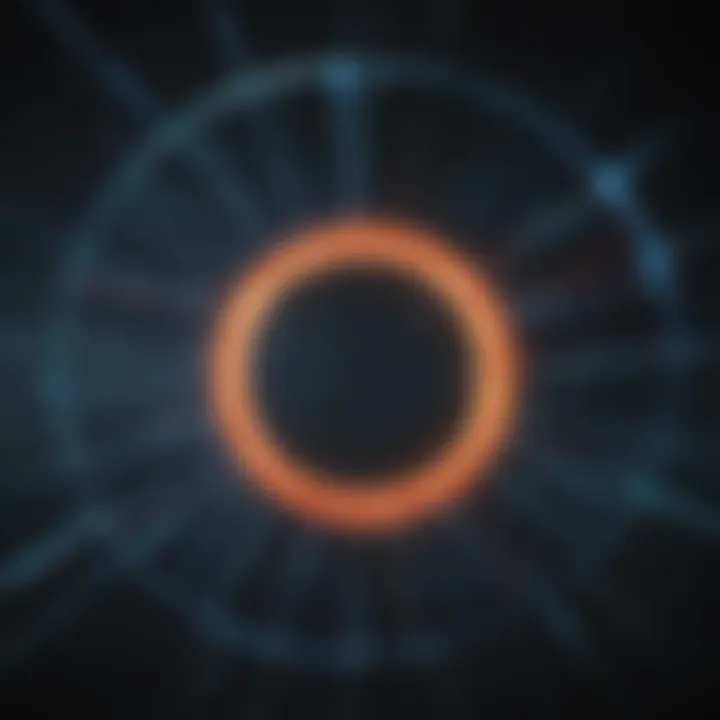
Intro
The realm of X-ray diffraction (XRD) offers a significant insight into the atomic structure of materials. A variety of scientific fields, including materials science, chemistry, and solid-state physics, leverage the principles of XRD to unveil characteristics at the microscopic level. Understanding how XRD operates provides a gateway for more profound inquiries into the materials we interact with daily.
X-ray diffraction is based on the interaction between x-rays and the electrons in a material. When an x-ray beam hits a crystalline substance, it scatters in various directions, producing a diffraction pattern that can be analyzed to infer detailed structural information about that material. This foundational principle is not just theoretical; its practical applications have expanded remarkably over the years.
In the sections that follow, we will explore the methodological approaches employed in XRD studies, alongside the implications this technology has across numerous disciplines. We will also discuss current trends shaping the future of XRD, highlighting innovative techniques and tools that enhance our understanding of complex materials.
Preface to X-ray Diffraction
X-ray diffraction (XRD) is an essential technique in materials science, chemistry, and physics. It provides insights into the structural properties of materials at the atomic level. Understanding the principles of X-ray diffraction is crucial for interpreting how materials interact with X-rays. This understanding can inform studies across various fields, including material characterization, crystallography, and nanotechnology.
XRD not only aids scientists in identifying the geometric arrangement of atoms within a crystal structure but also in characterizing materials' physical and chemical properties. Applications of XRD extend to the development of pharmaceuticals, electronics, and nanostructured materials. By grasping the core concepts and historical significance of X-ray diffraction, researchers can leverage these insights to advance in a multitude of scientific inquiries.
Moreover, exploring X-ray diffraction unveils its evolution over time. A historical context reveals how earlier methods laid the groundwork for today's sophisticated techniques, transforming the landscape of materials research. Recognizing this trajectory enhances the appreciation for ongoing advancements in XRD technology.
X-ray diffraction serves as a pivotal tool for scientists, enabling the detailed examination of crystal structures and their properties.
In summary, the rise of XRD has been significant, aligning perfectly with modern scientific challenges. The following sections endeavor to unravel its definition and examine its historical journey, thereby providing an informative foundation for discussions on the basic principles and applications that follow.
The Basic Principles of XRD
The principles of X-ray diffraction (XRD) are fundamental to understanding how this technique provides insight into the structure of materials. By grasping these principles, students, researchers, and professionals can recognize the underlying mechanisms that allow XRD to serve as a powerful tool for material characterization. The analysis of atomic arrangements and the resultant diffraction patterns hold significance in fields ranging from chemistry to materials science.
Understanding the basic principles can aid in better implementation and interpretation of XRD results, facilitating more accurate conclusions regarding material properties. Key concepts include the nature of X-rays and their interaction with crystalline structures. This knowledge is essential to optimize experimental setups and to troubleshoot common issues encountered in XRD analysis.
Nature of X-rays
X-rays are a form of electromagnetic radiation with wavelengths ranging from about 0.01 to 10 nanometers. This places them in a range suitable for probing atomic and molecular structures. Their high energy allows X-rays to penetrate many materials without causing significant damage, which makes them ideal for various applications, including medical imaging and materials characterization.
X-rays are produced when high-energy electrons collide with a metal target, often made of copper or molybdenum. This collision results in the emission of X-rays as the electrons lose energy. When X-rays interact with matter, they can either be absorbed or scattered. The scattering process is critical in XRD, enabling the determination of the crystal structure by analyzing the angles and intensities of the diffracted beams.
Diffraction and Interference
Diffraction is the bending of waves around obstacles and the spreading of waves when they pass through small apertures. When X-rays strike a crystalline material, they encounter regularly spaced atomic planes. According to the conditions set forth by Bragg's Law, constructive interference occurs when the path difference between waves reflected from successive planes is an integer multiple of the wavelength. This results in distinct peaks in the diffracted intensity that can be recorded and analyzed.
The interference pattern produced is characterized by peaks and troughs, which correspond to different angles of diffraction. Peaks in the XRD pattern represent constructive interference, indicating specific planes of atoms in the crystal lattice that are oriented favorably relative to the incoming beam of X-rays. Understanding this process is crucial for interpreting XRD data effectively.
Important Note: The intensity and position of these peaks contain information about the arrangement of atoms within the crystal, helping in phase identification and material characterization.
In summary, the principles of XRD provide the foundational knowledge necessary for engaging with the intricacies of material analysis. By comprehending the nature of X-rays and the principles of diffraction, one can better appreciate the capabilities and limitations of X-ray diffraction techniques.
The Physics Behind X-ray Diffraction
Understanding the physics behind X-ray diffraction is crucial for grasping how X-rays interact with matter. This interaction allows scientists to deduce structural information from materials at the atomic and molecular levels. Through X-ray diffraction, various properties of materials can be characterized, paving the way for advancements in multiple fields.
Bragg's Law forms the backbone of X-ray diffraction principles. This law articulates the conditions under which X-rays will constructively interfere when scattered by the lattice planes in crystals. Mathematically, it can be expressed as:
[ n\lambda = 2d\sin(\theta) ]
Where ( n ) is an integer, ( \lambda ) is the wavelength of the X-rays, ( d ) is the distance between lattice planes, and ( \theta ) is the angle of incidence. Understanding this equation helps researchers analyze the crystallographic structure effectively.
Another important aspect is the concept of crystal lattices and unit cells. A crystal lattice is a three-dimensional arrangement of atoms or molecules, while a unit cell is the smallest repeating unit of this lattice. The geometry of these structures determines how X-rays are scattered, influencing the appearance of XRD patterns.
In the context of material characterization, knowing how crystal lattices are formed and manipulated informs the design of new materials—especially in nanotechnology and pharmaceuticals. Here are several key points to consider regarding the physics of X-ray diffraction:
- X-rays penetrate deep into the material, allowing for bulk analysis.
- The wavelength of X-rays is comparable to the distance between atoms.
- The interaction of X-rays with matter produces unique diffraction patterns that reveal material structure.
"X-ray diffraction acts as a window into the atomic world, revealing intricate details about material properties that are otherwise invisible."
To sum up, the physics behind X-ray diffraction is foundational to the effective application of this technique. It enhances our understanding of material properties and their structural characteristics, making it an indispensable tool in research and industry.
Instrumentation in XRD
Instrumentation plays a critical role in X-ray diffraction (XRD) as it directly influences the quality and accuracy of the data obtained from the analysis. Various components of the XRD system function interactively to produce reliable diffraction patterns that can be interpreted for material characterization. A deep understanding of these instruments is essential for effectively utilizing XRD techniques in both research and industrial applications.
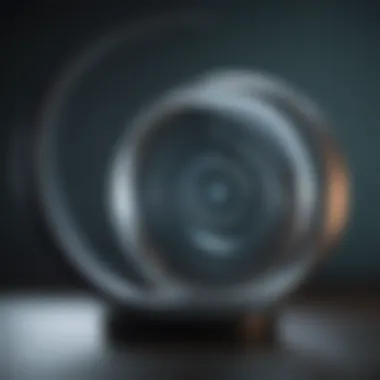
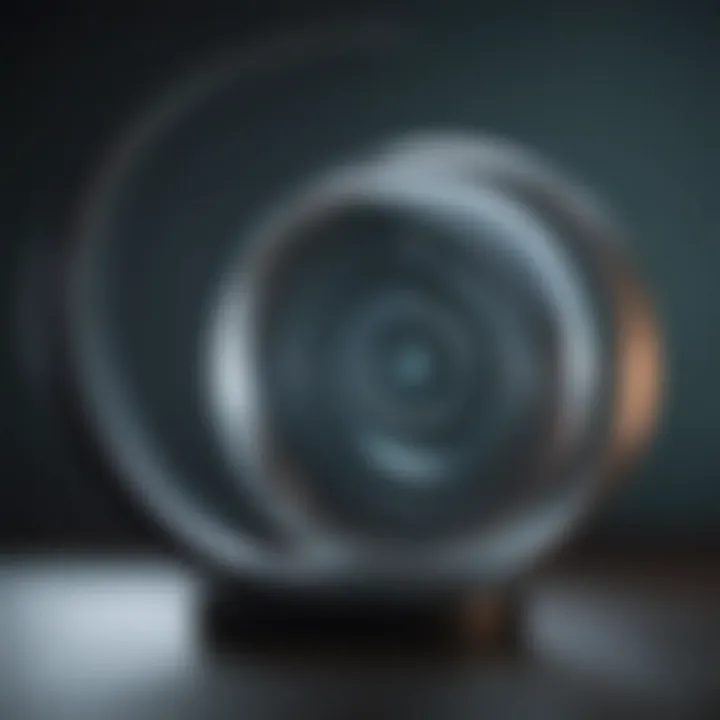
X-ray Source
The X-ray source is a fundamental part of any XRD system. Typically, X-ray tubes, rotating anodes, or synchrotron sources are employed, each with unique characteristics. X-ray tubes are common due to their cost-effectiveness and ease of use. They generate X-rays by bombarding a metal target, often copper or molybdenum, with electrons. This process produces characteristic X-ray radiation that is suitable for many applications.
Synchrotron sources offer higher intensity and brilliance, allowing for the analysis of weakly diffracting materials or small samples. They provide a highly collimated beam that enhances measurement precision; nevertheless, the requirement for a synchrotron facility limits their accessibility.
Choosing the appropriate X-ray source affects the resolution and intensity of the diffraction data. For instance, a higher energy source can penetrate denser materials, while lower-energy sources are ideal for softer substances. The design and operating conditions of the source also impact the time required for data collection, making this choice pivotal in experimental planning.
Detectors in X-ray Diffraction
Detectors convert the X-ray photons that are scattered off the sample into readable signals. In XRD, two broad categories of detectors are frequently utilized: point detectors and area detectors. Point detectors, such as scintillation counters, collect data at specific angles sequentially, which can be time-consuming. Area detectors, on the other hand, capture a two-dimensional array of data simultaneously. This is advantageous as it accelerates the data acquisition process while enhancing the overall accuracy.
Modern developments in detector technology have led to the adoption of complementary metal-oxide-semiconductor (CMOS) and pixel array detectors. These detectors offer superior sensitivity and clarity, thus improving the quality of diffraction patterns and enabling more detailed analyses. Factors like count rate, dynamic range, and energy resolution are key to selecting an appropriate detector. As X-ray diffraction techniques evolve, ongoing advancements in detector capabilities continue to enhance the scope of applications.
Sample Preparation Techniques
Proper sample preparation is essential for successful X-ray diffraction analysis. The quality of the sample directly influences the diffraction pattern obtained. Common techniques include grinding, which ensures the sample is finely powdered to maximize the surface area exposed to X-rays, and pelletizing, where the sample is compressed into disks.
For single crystal X-ray diffraction, samples must be of high purity, precisely oriented, and free of stress. Techniques such as solution growth or vapor diffusion might be employed to obtain suitable single crystals.
It is also essential to consider the alignment of the sample within the X-ray beam during preparation, as misalignment can lead to erroneous diffraction data. Moreover, various chemical treatments may be required to eliminate impurities or to enhance crystallinity.
In summary, ensuring optimal sample preparation and instrument alignment is paramount. Attention to detail at this stage can greatly support accurate and reproducible results, making it a critical aspect of the X-ray diffraction process.
Types of X-ray Diffraction Techniques
The study of X-ray diffraction (XRD) offers various techniques, each having distinct applications and advantages. These techniques provide valuable insights into the structural characteristics of materials. Understanding the types of X-ray diffraction techniques can guide researchers and professionals in selecting the appropriate method based on their specific requirements. The following sections outline three primary techniques: powder X-ray diffraction, single crystal diffraction, and high-resolution X-ray diffraction.
Powder X-ray Diffraction
Powder X-ray Diffraction (PXRD) is one of the most widely used techniques in the XRD family. It is particularly beneficial for analyzing polycrystalline samples, where the crystal grains are oriented randomly. The method works by diffusing X-rays through a finely powdered sample, which generates a diffraction pattern.
The importance of PXRD lies in its ability to determine phase composition and crystallographic properties. It enables the identification of different phases within a mixture using peak position and intensity analysis. This technique is crucial in material science, chemistry, and geology.
Some key points regarding Powder X-ray Diffraction include:
- Simplicity in sample preparation: The sample only needs to be ground to a fine powder, making it accessible for most laboratories.
- Quantitative analysis: PXRD can determine phase abundance, which is vital for material development.
- Non-destructive: Since the sample is not damaged during analysis, it can be used for further studies.
However, PXRD does have limitations. Sample size and quality can affect the accuracy of results. For accurate results, ensuring a homogeneous and representative sample is essential.
Single Crystal Diffraction
Single Crystal Diffraction (SCD) is another pivotal technique in the realm of X-ray diffraction. This method involves the analysis of a single crystal specimen, which must be well-formed and free from defects. SCD provides high-resolution data about the arrangement of atoms within the crystal lattice.
The advantages of this technique are noteworthy:
- Maximizes data quality: SCD can resolve more precise structural details compared to powder diffraction because the regular arrangement of atoms in a single crystal provides a clearer diffraction pattern.
- Determines absolute configurations: It can help ascertain stereochemistry of molecules, which is crucial in fields such as pharmacology.
SCD is extensively used in solid-state chemistry, crystallography, and material science. However, its main disadvantage is that preparing suitable single crystals can be a time-consuming and challenging process. The orientation of the crystal must also be aligned meticulously to obtain reliable results.
High-Resolution X-ray Diffraction
High-Resolution X-ray Diffraction (HRXRD) refers to techniques used to resolve small variations in lattice parameters and is particularly useful for studying thin films and heterostructures. HRXRD is known for its sensitivity in detecting small strains in the crystal lattice which can result from the growth or fabrication processes.
Its importance in research is highlighted by:
- Detailed information on structural integrity: HRXRD can help understand defects or dislocations within materials, which is essential for the development of new materials.
- Application in semiconductor research: It plays a vital role in analyzing semiconductor materials crucial for electronics and photonics.
Even though HRXRD provides advanced information, one must consider its complexity in instrumentation and data analysis. This technique often requires specialized equipment and expertise.
High-Resolution X-ray Diffraction is an essential method for analyzing semiconductor materials, providing critical insights into their structural integrity.
Applications of X-ray Diffraction
X-ray diffraction (XRD) serves multiple roles in scientific research and industrial processes. It plays a crucial part in identifying crystalline materials and determining material properties. The implications of XRD are far-reaching, impacting sectors from chemistry to material sciences. Understanding its applications can illuminate its value in advancing technology and research.
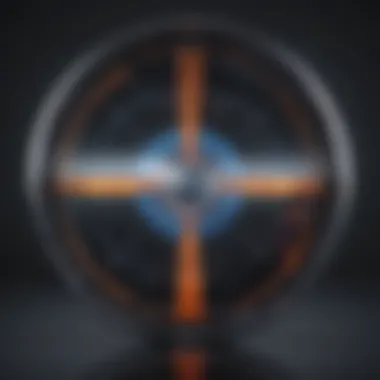
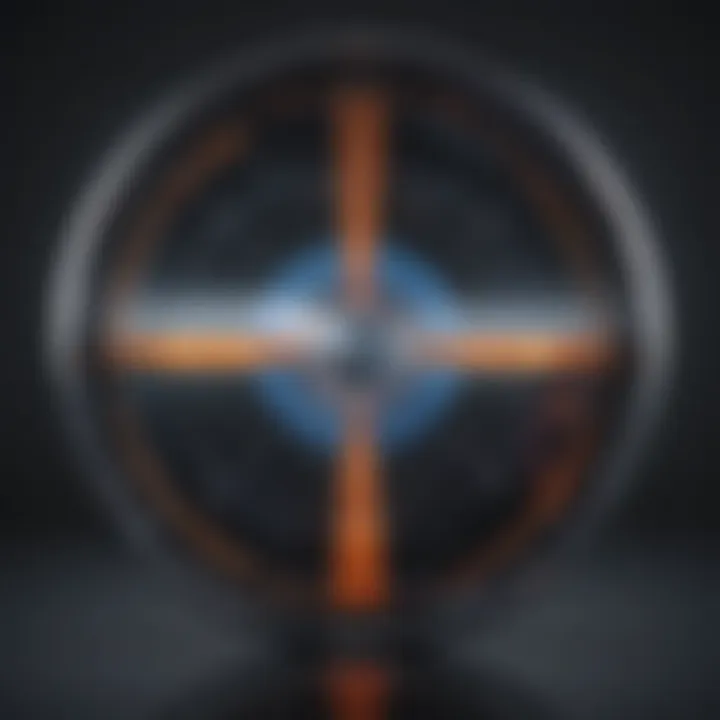
Material Characterization
Material characterization is one of the primary applications of X-ray diffraction. XRD enables precise analysis of a material's structure. It helps in identifying the arrangement of atoms within a crystal lattice, which is vital in determining the physical properties of the material.
The significance of material characterization extends to various fields. For instance, in metallurgy, XRD identifies phase transformations that occur during the processing of alloys. In geology, it assists in the identification of minerals, which can guide resource extraction operations. This use of X-ray diffraction underscores its role in research and development, as well as quality control processes.
X-ray diffraction allows researchers to evaluate:
- Crystallinity: Assessing how well-ordered a material is, which affects properties like strength and conductivity.
- Phase composition: Determining the various phases present in a sample and their relative proportions.
- Texture: Understanding the orientation of crystals within a sample, which is essential in materials science.
Pharmaceutical Applications
In the pharmaceutical industry, X-ray diffraction is essential for drug development and quality assurance. It is used to analyze the crystalline structure of drug compounds, influencing their solubility and bioavailability. The identification of polymorphs is crucial, as different forms of the same compound can exhibit significantly varied properties.
The role of XRD in pharmaceuticals also extends to:
- Stability studies: Determining how changes in temperature or humidity may affect the crystalline structure and stability of drugs.
- Formulation development: Optimizing formulations to ensure consistency in quality and performance.
By providing critical insights into the structural characteristics of drug compounds, X-ray diffraction enhances the effectiveness and safety of pharmaceuticals.
Nanotechnology and Beyond
Nanotechnology is one of the most promising fields where X-ray diffraction finds its applications. At the nanoscale, materials exhibit different properties compared to their bulk counterparts. XRD helps characterize nanomaterials, helping scientists understand how their structural characteristics influence their function.
The applicability of X-ray diffraction in nanotechnology includes:
- Nanostructure assessment: Analyzing size, shape, and distribution of nanoparticles, which are critical for applications in electronics, medicine, and catalysis.
- Thin film analysis: Understanding the structural properties of thin films used in electronic devices and photovoltaic cells.
X-ray diffraction is also finding applications beyond traditional boundaries. Researchers explore its use in developing materials for renewable energy, battery technology, and advanced photonic devices, thus pushing the limits of scientific investigation and technological innovation.
Understanding XRD Patterns
Understanding XRD patterns is a crucial aspect of analyzing materials using X-ray diffraction. These patterns provide rich information about the atomic structure of crystalline materials. By interpreting these patterns, researchers can gain insights into the material's properties, such as phase composition, crystallinity, and internal structure. XRD patterns consist of peaks that represent constructive interference of X-rays scattered by the crystal lattice. Each peak corresponds to specific planes in the crystal and carries information about the arrangement of atoms.
This topic is significant for several reasons. First, it allows scientists to identify unknown materials by comparing observed patterns with databases of known structures. Second, intensity analysis reveals details about the proportions of different phases present in the sample. Third, examining the width and position of the peaks helps in understanding stress, strain, and defects within the material.
In summary, understanding XRD patterns provides a window into the internal architecture of materials, enabling advancements in numerous fields, including materials science, geology, and chemistry.
Peaks and Intensity Analysis
Peaks in XRD patterns are pivotal for characterizing materials. Each peak's position, represented as an angle or 2θ value, indicates the spacing of crystal planes according to Bragg's Law. The number and position of these peaks help ascertain the phase of the material. If there are several peaks, this can signify a multi-phase sample.
The intensity of these peaks is equally important. It relates to the number of atoms in the unit cell and the structure factors of the planes involved. Stronger peaks imply a higher concentration of sin the sample, indicating that materials have ordered structures, while broader peaks may denote a more amorphous or disordered arrangement. Understanding these characteristics allows researchers to differentiate between similar materials or phases effectively.
Key Considerations:
- Peak positions provide information about crystal structure.
- Peak intensities relate to atomic density and arrangement.
- Analyzing peak shapes can hint at defects or size effects in crystals.
Phase Identification
Phase identification involves determining the specific crystalline phases within a material based on its XRD pattern. Each unique crystalline structure has a distinct diffraction pattern. Thus, comparing the obtained pattern with reference databases, such as the International Center for Diffraction Data (ICDD), facilitates the identification process.
Phase identification is critical in various applications. In material science, it helps in evaluating the performance of alloys or polymers. In pharmaceuticals, it can determine the polymorphic forms of a compound, impacting solubility and bioavailability. Accurate phase IDs can also be essential in geosciences for characterizing minerals in rocks or soils.
Effective phase identification saves both time and resources in research and industry. It ensures that materials used are optimized for their intended application, minimizing trial and error in material studies.
Limitations of X-ray Diffraction
X-ray diffraction (XRD) is a powerful tool in material characterization; however, it comes with various limitations that can affect its application and effectiveness. Understanding these limitations is crucial for researchers and professionals in the field, as they influence experimental design and data interpretation. This section will explore two prominent limitations: sample size and quality, and elemental sensitivity.
Sample Size and Quality
The size and quality of the sample used in X-ray diffraction play a significant role in obtaining accurate results. For effective diffraction, a sample must be homogenous and adequately sized. If the sample is too small, the X-rays may scatter unevenly, leading to weak or indistinct diffraction patterns. Conversely, large samples can introduce complications, such as texture and strain, which can distort results.
Sample quality encompasses its purity and crystallinity. Impurities or amorphous regions within a sample can result in background noise, making it difficult to resolve significant peaks in the diffraction pattern. Furthermore, poorly crystallized samples may lead to broadening of the diffraction peaks, complicating phase identification. In the context of research and industrial applications, the limitations in sample size and quality often necessitate careful sample preparation techniques to ensure reliable and reproducible results.
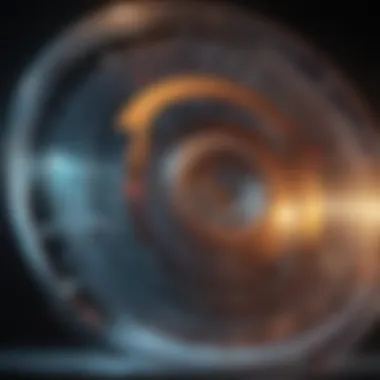
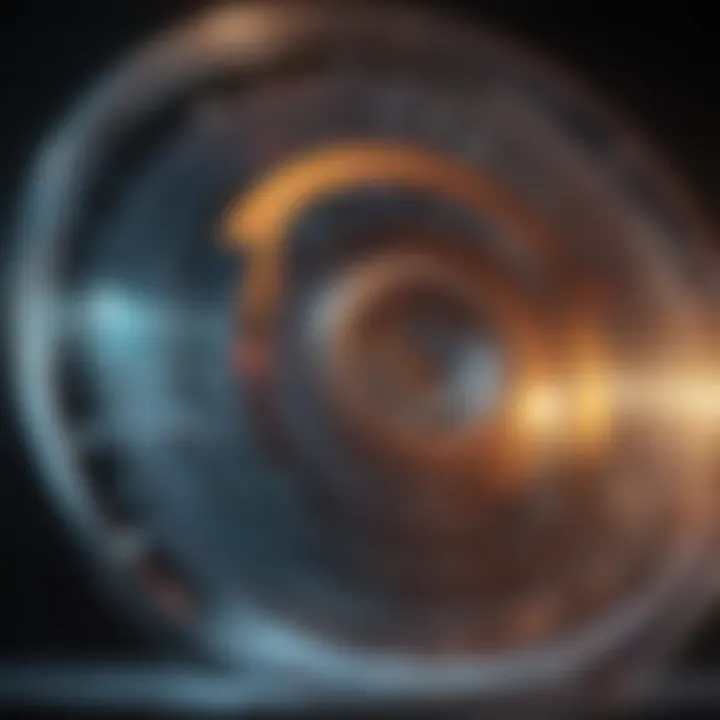
Elemental Sensitivity
Elemental sensitivity refers to the capability of X-ray diffraction to distinguish between different elements within a sample. XRD is generally more effective for heavier elements, as they produce more distinct diffraction peaks. In contrast, lighter elements, such as lithium or beryllium, often result in weaker signals that may be indistinguishable from background noise. This limitation hinders the comprehensive analysis of materials that are low in heavier elements or composed predominantly of lighter counterparts.
Moreover, overlapping diffraction peaks can occur when elements present in the sample are spectrally close. This overlap complicates the analysis, potentially leading to inaccuracies in quantifying phases present and misidentifying materials. Understanding these limitations helps practitioners interpret results within the proper context, ensuring accurate conclusions are drawn from X-ray diffraction studies.
Effective use of X-ray diffraction necessitates awareness of its limitations regarding sample size and elemental sensitivity.
Recent Advancements in XRD Technology
Recent advancements in X-ray diffraction (XRD) technology have notably transformed the landscape of material science and related fields. These developments not only enhance the efficiency of diffraction techniques but also expand their range of applications. Understanding these advancements is crucial for researchers and professionals who are involved in material characterization and analysis.
Synchrotron X-ray Sources
Synchrotron X-ray sources represent a significant leap in XRD technology. These facilities generate highly intense and coherent X-ray beams, which allow for more precise measurements of diffraction patterns. The key advantage of synchrotron sources is their ability to produce X-rays with tunable wavelengths.
The tunability enables scientists to select the optimal X-ray energy for specific experiments, facilitating detailed studies of complex materials. For instance, synchrotron radiation can penetrate samples that conventional X-ray sources cannot, thus revealing hidden crystal structures. Moreover, the high intensity improves the signal-to-noise ratio in experiments, leading to more reliable results. Recent developments in this area have involved the construction of new synchrotron facilities and enhancements to existing technologies, making XRD more accessible to researchers worldwide.
Innovations in Detector Technology
Advancements in detector technology are also pivotal in enhancing XRD analysis. New types of detectors, such as pixelated detectors and photon-counting detectors, are being implemented in laboratories globally. These detectors are known for their superior speed and sensitivity, which results in faster data acquisition and higher precision in measurement.
For example, a modern detector can process thousands of counts per second, allowing for real-time monitoring of dynamic processes within materials. Furthermore, improved software algorithms associated with these detectors enhance data analysis, enabling better peak identification and quantification.
The integration of advanced detectors into XRD setups not only improves resolution but also increases the overall effectiveness of the X-ray diffraction technique. By combining these new technologies with established methods, researchers can gain detailed insights into material properties, which can lead to innovations in various fields, including nanotechnology and pharmaceuticals.
The evolution of detector technology and synchrotron sources symbolizes a new era in X-ray diffraction, opening doors to extensive research possibilities and innovative applications.
Future Directions in X-ray Diffraction Research
In recent years, X-ray diffraction (XRD) has shown significant promise, not only in material science but also in interdisciplinary fields. Understanding future directions in XRD research is paramount for advancing both practical applications and theoretical knowledge. This section will delve into particular areas ripe for exploration, evaluating their potential benefits and the necessary aspects of development.
Integrating Multiscale Techniques
Integrating multiscale techniques is essential for enhancing the precision and functionality of X-ray diffraction studies. By combining different imaging scales, researchers can interactively study material properties from atomic to macroscopic levels. This approach allows for a more holistic understanding of material behavior under various conditions.
For example, coupling X-ray diffraction with other techniques such as electron microscopy or nuclear magnetic resonance can yield comprehensive insights. This synergy enables the characterization of complex structures and can reveal phase transitions that are not just visible under a single method. The main benefits of this integration include:
- Improved accuracy: More data points lead to enhanced reliability in results.
- In-depth analysis: Allows for keen detection of subtle structural changes.
- Broader applicability: Expands potential applications across various domains like nanotechnology and pharmaceuticals.
However, the integration poses challenges. Researchers must address instrumentation compatibility and the data interpretation complexity involved.
Application in Emerging Fields
As industries evolve, the application of X-ray diffraction in emerging fields is becoming increasingly relevant. Fields such as nanotechnology, biomedicine, and renewable energy greatly benefit from the techniques offered by XRD. The adaptability of X-ray diffraction techniques is crucial to meet the changing demands of these new areas.
In nanotechnology, for instance, XRD is pivotal for material characterization at the nanoscale, assisting in the development of novel nanomaterials. The precision in determining crystal structures aids in the synthesis of materials with desired properties. In biomedicine, XRD enables the study of complex biomolecules, providing insight into protein structures and aiding drug design.
Some notable applications include:
- Nano-structured materials: Evaluating dimensions and crystallographic particulars which influence the properties of the materials.
- Drug formulation: Understanding polymorphism in drug compounds can optimize effectiveness and stability.
- Energy materials: Assessing the structural integrity of materials used in energy storage devices enhances efficiency.
In summary, the future of X-ray diffraction research holds exciting possibilities for integration and application in various sectors. The continued development of techniques will provide more accurate results and open doors to innovative research paths. The focus on collaborative methods and interdisciplinary practices will further enrich the field, ensuring that XRD remains a key player in scientific advancement.
Closure
The conclusion of this article underscores the significant impact and relevance of X-ray diffraction (XRD) in the realm of scientific inquiry and material science. As we have explored throughout the previous sections, XRD offers a unique lens through which to understand the crystalline structures and properties of various materials. By interpreting diffraction patterns, researchers can unlock vital information that influences everything from materials engineering to pharmaceutical development.
Summary of Key Concepts
In synthesizing the key concepts discussed, it is clear that X-ray diffraction is not solely a technique but a fundamental tool that enables scientists to probe the atomic arrangement within materials. The principles behind X-ray diffraction, notably Bragg's Law and the interaction between X-rays and crystal lattices, lay the groundwork for practical applications in material characterization. Key points include:
- The essential understanding of X-ray behavior.
- The methodologies employed in constructing accurate diffraction patterns.
- The role of contemporary advancements, particularly synchrotron X-ray sources and novel detector technologies.
This consolidation of knowledge serves to equip students, researchers, and professionals alike with a comprehensive understanding of how XRD operates within its scientific framework.
The Future of X-ray Diffraction
Looking ahead, the trajectory of X-ray diffraction is poised for continued evolution. Emerging fields, including nanotechnology and materials science, promise to expand the applicability of XRD techniques. Future research will likely integrate multiscale approaches, merging XRD with other characterization methods, enhancing the depth of material analysis. Considerations include:
- Development of more advanced detector technologies.
- Increased use of data analytics and machine learning for pattern interpretation.
- Broader application in interdisciplinary fields, such as biomedical materials.
The future holds exciting potential as researchers refine XRD applications and methodologies, further establishing its impact on both academia and industry. The ongoing dialogue between emerging scientific needs and traditional diffraction techniques presents an enriching opportunity for those in the field.